This blog post is made for practising structural engineers in the field of the telecom industry or others who design tall structures exposed to wind. I will go through a procedure step-by-step how to establish wind loads on telecom antennas of a rooftop mast in practise.
By "practical", I mean a practical guide and not necessarily the procedure what EC suggest to use. As practising engineers we like to be efficient and spend less time on eventually less productive tasks. However, when it comes to the European designs code series, we can say a lot, but not practical in certain areas. You may judge this statement but comparing with, for instance, ASCE design code series, or in the telecom industry's TIA-222 codes, it provides leaner solutions and far less complicated design procedures. Probably in a later blog I'll make a step-by-step comparison between the two codes. By the end of this post you will understand what steps need to be made to establish the proper wind forces on the antennas. The guide will heavily be referenced with the design code chapters of the code, so it requires that you are familiar with the EN 1991-1-4. The calculation will pick up some values from the national annex of the Netherlands, but those can be replaced by any of the European country national values to fit your case.
I tried my best to explain all steps but in case I missed something or steps are not clear, please drop me a question at the end of the blog at the Comments section.
Introduction
The telecom mast in our case will be a single rooftop pole, braced by two diagonals and the structure is supported on a H-beam frame. See the FEA model as below. A linear static analysis including buckling and a dynamic analysis were performed.

Input data
Environmental parameters
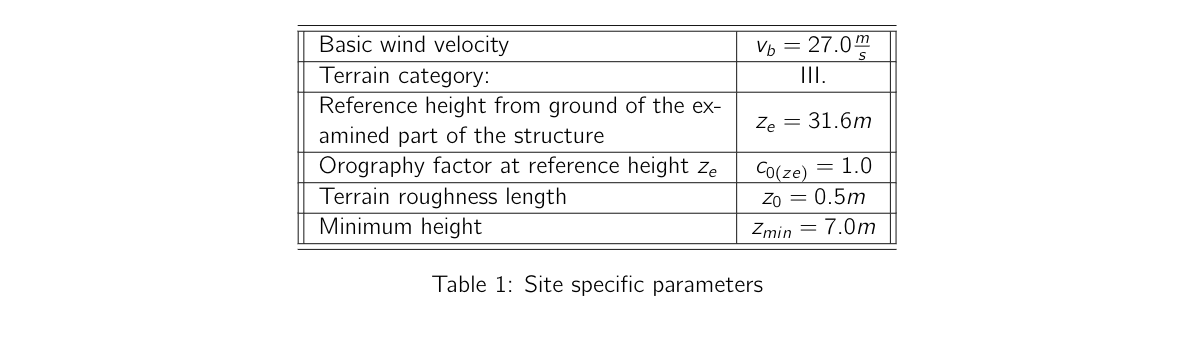
Nationally Defined Parameters
Air density: $\rho = 1.25 \frac{kg}{m^3}$
Calculation of peak velocity pressure
Basic wind velocity
The basic wind velocity $v_b$ is defined in EN1991-1-4 §4.2(2)P as a
function of the wind direction and time of year at 10 m above ground of
terrain category III. It is calculated as:
$$v_b = c_{dir} \cdot c_{season} \cdot v_{b,0}$$
where $v_{b,0}$ is the fundamental value of the basic wind velocity which is defined in EN1991-1-4 §4.2(1)P as the characteristic 10 minutes mean wind velocity at 10 m above ground level for terrain category III. The value of $v_{b,0}$ is provided in the National Annex based on the climatic conditions of the region where the structure is located. The influence of altitude on the basic wind velocity $v_{b}$ has been also specified in the National Annex.
The directional factor $c_{dir}$ and the seasonal factor $c_{season}$ are defined in EN1991-1-4 §4.2(2)P and they take into account the effects of wind direction and time of the year. Their values are generally equal to $c_{dir}$ = 1.0 and $c_{season}$ = 1.0. The National Annex may specify values of $c_{dir}$ and $c_{season}$ different than 1.0.
In the following calculations the basic wind velocity is considered as $v_b = 27 \frac{m}{s}$.
Terrain roughness
The roughness length $z_o$ and the minimum height $z_min$ are specified in EN1991-1-4 Table 4.1 as a function of the terrain category. For terrain category III. the corresponding values are $z_0 = 0.50m$ and $z_{min} = 7.0m$.
The terrain factor $k_r$ depending on the roughness length $z_0 = 0.50m$ is calculated in accordance with EN1991-1-4 equation (4.5):
$$k_r = 0.19 \left(\frac{z_0} {z_{0,II}} \right)^{0.07} = 0.19 \left( \frac{0.5 m}{0.05m} \right)^{0.07} = 0.223$$
The roughness factor $c_r(z_e)$ at the reference height $z_e$ accounts for the variability of the mean wind velocity at the site of the structure due to the height above ground level and the ground roughness of the terrain upwind of the structure. It is calculated in accordance with EN1991-1-4 equation 4.4.
For the case where $z_e \geqq z_{min}$:
$$c_r(z_e) = k_r \cdot ln(\frac{z_e}{z_o}) = 0.223 \cdot \ln \left( \frac{31.6m}{0.5m} \right) = 0.926$$
Orography factor
Where orography (e.g. hills, cliffs etc.) increases wind velocities by more than 5% the effects should be taken into account using an orography factor $c_0(z_e)$ different than 1.0, as specified in EN1994-1-1 §4.3.3. In general the effects of orography may be neglected when the average slope of the upwind terrain is less than 3° up to a distance of 10 times the height of the isolated orographic feature. The recommended procedure in EN1994-1-1 §4.3.3 for calculation of the orography factor $c_0(z_e)$ is described in EN1994-1-1 §A.3.
In the following calculations the orography factor is considered as $c_0(z_e) = 1.000$
Mean wind velocity
The mean wind velocity $v_m(z_e)$ at reference height $z_e$ depends on the terrain roughness, terrain orography and the basic wind velocity vb. It is determined using EN1991-1-4 equation (4.3):
$$v_m(z_e) = c_r(z_e) \cdot c_0(z_e) \cdot v_b = 0.926 \cdot 1.000 \cdot 27.00 \frac{m}{s} = 24.99 \frac{m}{s}$$
Wind turbulence
The turbulence intensity $I_v(z_e)$ at reference height $z_e$ is defined as the standard deviation of the turbulence divided by the mean wind velocity. It is calculated in accordance with EN1991-1-4 equation 4.7.
For the case where $z_e \geqq z_{min}$: $$
I_v(z_e) = \frac{k_I}{ c_0(z_e) \cdot \ln \left( \frac{z_e}{z_0} \right)} $$
so,
$$I_v(z_e) = \frac{1.0}{ 1.0 \cdot \ln \left( \frac{31.6m}{0.5m} \right)} = 0.241$$
where the turbulence factor is considered as $k_I = 1.000$ in accordance with EN1991-1-4 §4.4(1).
Basic velocity pressure
The basic velocity pressure $q_b$ is the pressure corresponding to the wind momentum determined at the basic wind velocity $v_b$. The basic velocity pressure is calculated according to the following fundamental relation, as specified in EN1991-14 §4.5(1):
$$q_b = \frac{1}{2} \cdot \rho \cdot v_b^2 = \frac{1}{2} \cdot 1.25 \frac{kg}{m^3} \cdot 27.0^2 \frac{m}{s^2} = 0.456 \frac{kN}{m^2}$$
where $\rho$ is the density of the air in accordance with EN1991-1-4 §4.5(1). In this calculation the following value is considered:
$\rho = 1.25 \frac{kg}{m^3}$.
Peak velocity pressure
The peak velocity pressure $q_p(z_e)$ at reference height $z_e$ includes mean and short-term velocity fluctuations. It is determined according to EN1991-1-4 equation 4.8:
$$q_p(z_e) = \left[ 1+7 \cdot I_v(z_e) \right] \cdot \frac{1}{2} \cdot \rho \cdot v_m^2$$
then,
$$q_p(z_e) = \left[ 1+7 \cdot 0.241 \right] \cdot \frac{1}{2} \cdot 1.25 \cdot 24.99^2 = 1.049 \frac{kN}{m^2}$$
where the density of the air is considered as $\rho = 1.25 \frac{kg}{m^3}$ in accordance with EN1991-1-4 §4.5(1).
The calculated value of $q_p(z_e)$ corresponds to an exposure factor $c_e(z_e)$:
$$c_e(z_e) = \frac{ q_p(z_e) }{q_b} $$
so,
$$ c_e(z_e) = \frac{ 1.049 }{0.456} = 2.300$$
Therefore the peak velocity pressure is calculated as $q_p(z_e) = 1.049\frac{kN}{m^2}$.
Calculation of wind forces and pressures on the structure
The wind actions on the structure (forces and pressures) depend on $q_p(z_e)$ as follows.
Wind pressures on surfaces
The wind pressure on surfaces are derived from the calculated value of $q_p(z_e) = 1.049 kN/m2$ by application of the appropriate pressure coefficient, as specified in EN1991-1-4 §5.2.
For external surfaces the applicable wind pressure $w_e$ is calculated as:
$$w_e = q_p(z_e) \cdot c_{pe}$$
where $c_{pe}$ is the appropriate pressure coefficient for external pressure that is given in EN1991-1-4 Section 7 depending on the type of structure. The appropriate reference height $z_e$ for the external surface is given in EN1991-1-4 Section 7 depending on the type of structure.
For internal surfaces the applicable wind pressure $w_i$ is
calculated as: $$w_i = q_p(z_i) \cdot c_{pi}$$
where $c_{pi}$ is the appropriate pressure coefficient for internal pressure that is given in EN1991-1-4 Section 7 depending on the type of structure. The appropriate reference height $z_i$ for the internal surface is given in EN1991-1-4 Section 7 depending on the type of structure.
Total wind force on structure or structural element
The overall wind effect on the total structure or a particular structural element is expressed by the total wind force Fw that is estimated by application of the appropriate force coefficient, as specified in EN1991-1-4 §5.3.
$$F_w = c_sc_d \cdot c_f \cdot q_p(z_e) \cdot A_{ref}$$
where:
The structural factor $c_sc_d$ takes into account the structure size effects from the non-simultaneous occurrence of peak wind pressures on the surface and the dynamic effects from the effect of structural vibrations due to turbulence. The structural factor $c_sc_d$ is determined in accordance with EN1991-1-4 Section 6. A value of $c_sc_d$ = 1.0 is generally conservative for small structures not-susceptible to wind turbulence effects such as buildings with height less than 15 m.
Structural factor $c_sc_d$
Normative procedure
According to the National Annex of NEN-EN 1991-1-4 Annex C as Procedure (2) are normative to use when establishing the $c_sc_d$ structural factor. However, the turbulence should be calculated according to B.1.
$$c_s = \frac{1+7 \cdot I_v(z_s) \cdot \sqrt{B^2}}{1+7 \cdot I_v(z_s)}$$
while
$$c_d = \frac{1+2 \cdot k_p \cdot I_v(z_s) \cdot \sqrt{B^2+R^2} }{1+7 \cdot I_v(z_s) \cdot \sqrt{B^2}}$$
The procedure can only be established if our structure correspond to one of the general shapes shown in the below figure.

The turbulent length scale
The turbulent length scale $L_z$ represents the average gust size for natural winds. For heights $z$ below 200m the turbulent length scale may be calculated using the following expression. In cases when
$z \geqq z_{min}$:
$$L_{(z_s)} = L_t \cdot \left( \frac{z}{z_t} \right) ^\alpha$$
with a reference height of $z_t = 200m$, a reference scale of $L_t = 300m$, and with $\alpha = 0.67 + 0.05 \cdot \ln(z_0)$.
$$L_{(z_s)} = 300 \cdot \left( \frac{3.4}{200} \right) ^{0.67+0.05 \cdot \ln{0.5}}
$$
so,
$$ L_{(z_s)} = 23.0m$$
Structural factor
The structural factor $c_sc_d$ is defined in 6.3.1. The background factor $B^2$ allowing for the lack of full correlation of the pressure on the structure surface may be calculated using the following expression:
$$B^2 = \frac{1}{1+\frac{3}{2} \cdot \sqrt{ \left( \frac{b}{L(z_s)^2} \right) + \left( \frac{h}{L(z_s)^2} \right) + \left( \frac{b}{L(z_s)^2} \cdot \frac{h}{L(z_s)^2} \right) } }$$
In case $b=1.4m$ and $h=2.8m$ this yields to: $$B^2 = 0.828$$
The resonance response factor
$R^2$ allowing for turbulence in resonance with the considered vibration mode of the structure should be determined using the following expression:
$$R^2 = \frac{\pi^2}{2 \cdot \delta } \cdot S_L(z_s,n_{1,x}) \cdot K_s \cdot (n_{1,x})$$
The size reduction function Ks may be approximated by the following expression:
$$K_s(n) = \frac{1}{1+ \sqrt{ (G_y\cdot \phi_y)^2 + (G_z\cdot \phi_z)^2 + \left( \frac{2}{\pi} \cdot G_y \cdot \phi_y \cdot G_z\cdot \phi_z \right)^2 } } \label{eq:Ks_n} $$
then,
$$ \phi_y = \frac{c_y \cdot b \cdot n}{v_m \cdot (z_s) } $$
and,
$$ \phi_z = \frac{c_z \cdot h \cdot n}{v_m \cdot (z_s) }$$
The constants $G_y$ and $G_z$ depend on the mode shape variation along the horizontal y-axis and vertical z-axis, respectively. The decay constants $c_y$ and $c_z$ are both equal to 11,5. The constant G introduced in below equation and the constant K used to calculate accelerations, are shown in Table C.1. A roof-top mast (similar to chimneys) has a uniform horizontal mode shape variation and a parabolic vertical mode shape variation $G_y = \frac{1}{2}$, $G_z = \frac{5}{18}$, $K_y = 1$ and
$K_z = \frac{5}{3}$. From here:
$$\phi_y = \frac{11.5 \cdot 1.4 \cdot 3.177}{24.99 \cdot (3.4) } = 0.602 $$
and,
$$\phi_z = \frac{11.5 \cdot 2.8 \cdot 3.177}{24.99 \cdot (3.4) } = 1.204 $$
then,
$$ K_s(n) = \frac{1}{1+ \sqrt{ (\frac{1}{2}\cdot 0.602)^2 + (\frac{5}{18}\cdot 0.1.204)^2 + \left( \frac{2}{\pi} \cdot \frac{1}{2} \cdot 0.602 \cdot \frac{5}{18} \cdot 1.204 \right)^2 } } $$
finally,
$$ K_s(n) = 0.688$$
The wind distribution over frequencies is expressed by the non-dimensional power spectral density function $S_L(z,n)$, which should be determined using the following expression:

$$f_L(z,n) = \frac{n \cdot L(z)}{v_m(z)} = \frac{3.177 \cdot 23}{24.99} = 2.924$$
Where $f_L(z,n)$ is a non-dimensional frequency determined by the frequency $n=n_{1,x}$ the natural frequency of the structure in Hz, by the mean velocity $v_{m(z)}$ and the turbulence length scale $L(z)$ defined in (B.1). The power spectral density function is illustrated in the figure above. For the dynamic system I have applied nodal masses to the structure at the fixtures of the panel antennas. The mass distribution is 70% of the total mass applied at the upper part and 30% at the lower mounting point.The applied masses are 187kg and 56kg respectively. See dynamic results on the image below. Lowest Eigen-frequency mode found at 3.177Hz.
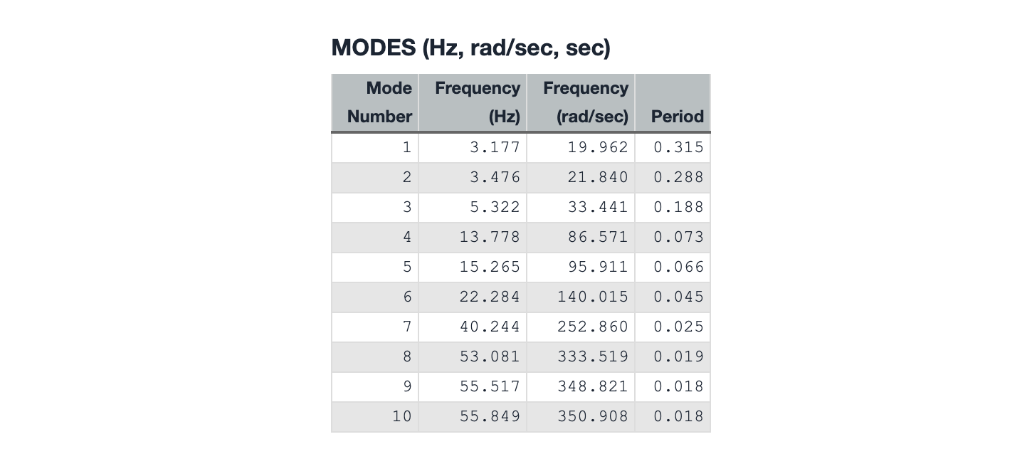
By using the Power spectral density graph in the diagramm above $S_L(z,n)$ will yield to $\approx 0.07$. Finally the response factor $R^2$ should be determined by the following expression:
$$R^2 = \frac{\pi^2}{2 \cdot \delta} \cdot S_L(z_s,n_{1,x}) \cdot K_s \cdot (n_{1,x})$$
The logarithmic decrement of damping $\delta$ for fundamental bending mode
may be estimated by the following expression.
$$\delta = \delta_s+\delta_a+\delta_d$$
We do not have any special damping device, so the formula might be simplified to:
$$\delta = \delta_s+\delta_a$$
One of the factors worth mentioning here is the equivalent mass per elevational area, $\mu_e$, which has to be estimated for the structure at hand. Under certain criteria, $\mu_e$ could be replaced by the equivalent mass per area, $m_e$. According to § F.4(2), $m_e$ could be represented by the average mass per meter over the top third of the structure. $n_{1,x}$ represents the frequency of the lowest Eigen-mode, which could be estimated by §F.2(2). $\delta_s = 0.02$ can be estimated from Table (F.2) With this at hand:
$$\delta_a = \frac{c_f \cdot \rho \cdot b \cdot v_m(z_s)}{2 \cdot n_1 \cdot m_e} $$
so,
$$\delta_a = \frac{1.3 \cdot 1.25 \cdot 1.4 \cdot 24.99}{2 \cdot 3.177 \cdot 100} = 0.089$$
and finally:
$$\delta = 0.02 + 0.089 = 0.109$$
From here we can calculate $R^2$ as follows:
$$R^2 = \frac{\pi^2}{2 \cdot \delta} \cdot S_L(z_s,n_{1,x}) \cdot K_s(n_{1,x}) $$
so,
$$R^2 = \frac{\pi^2}{2 \cdot 0.109} \cdot 0.07 \cdot 0.688 = 2.108$$
Then back to our basic formulas for $c_s$ and $c_d$:
$$c_s = \frac{1+7 \cdot I_v(z_s) \cdot \sqrt{B^2}}{1+7 \cdot I_v(z_s)} $$
so,
$$c_s = \frac{1+7 \cdot 0.241 \cdot \sqrt{0.828}}{1+7 \cdot 0.241} = 0.94$$
while
$$c_d = \frac{1+2 \cdot k_p \cdot I_v(z_s) \cdot \sqrt{B^2+R^2} }{1+7 \cdot I_v(z_s) \cdot \sqrt{B^2}} $$
finally,
$$c_d = \frac{1+2 \cdot 3.64 \cdot 0.241 \cdot \sqrt{0.828+2.108} }{1+7 \cdot 0.241 \cdot \sqrt{0.828}} = 1.59$$
The computed $c_sc_d$ factor
$$c_sc_d = 0.94 \cdot 1.59 = 1.49$$
Force coefficient
The force coefficient $c_f$ is given in EN1991-1-4 Sections 7 and 8 depending on the type of structure or structural element. The wind force Fw acting on a structure or a structural component may be determined by vectorial summation over the individual structural elements (as shown in 7.2.2) by using the force coefficient formula,
$$F_w=c_sc_d \cdot \sum_{elements} c_f \cdot q_p(z_e) \cdot A_{ref}$$
Structure
The force coefficient, $c_f$, of lattice structures and scaffolding with parallel chords will be obtained by the following expression:
$$c_f = c_{f,0} \cdot \psi_{\lambda}$$ I'll not go into details of the force coefficient calculations of the each strutural members, but the values were computed by taking into account slenderness, Reynolds numbers, end-effects and solidity ratios. We also implement $c_{prob}$ for a shorter Design working life as 15 years instead of 50, demonstrating the impact of $c_{prob}$.
Antennas
I'm using force values here from a typical 5G antenna data sheet in 3 directions exposed to wind, as front, side and back. The basic idea here is to derive the $c_f$ values from the each direction to wind the antenna is exposed to, based on the wind forces under the lab conditions. In this case the wind tunnel tests were performed at $150\frac{km}{h}$. The individual force coefficient values then can be used to determine wind forces on the antennas at any given peak velocity. The formula to calculate the $c_f$ values for the antennas (Table 3.) is the following:
$$ c_f = \frac{F_{w(150)}}{\frac{1}{2} \cdot \rho \cdot v^2 \cdot A_{ref} } $$

Antenna configurations
I'll demonstrate two scenarios for antenna configurations. First, when we have 3 sector antennas, and second with 2 antennas involved.
3-antenna configuration
Eurocode doesn't suggest any exact method how to calculate wind pressures on the antenna appurtenances. We can use an established method from TIA-222. The basic concept is that we calculate the effective projected areas for normal and side face of the antennas, $EPA_N$ and $EPA_T$ respectively. TIA code suggest the following formula for computing the total EPA of the appurtenances.
$$(EPA)_A = K_a[(EPA)_N \cdot cos^2(\theta)+(EPA)_T \cdot sin^2(\theta)]$$
where $\theta$ is the relative angle between the azimuth associated with
the normal face of the appurtenance and the wind direction. EPA, effective
projected area means simply the antenna area times the associated force
coefficients.
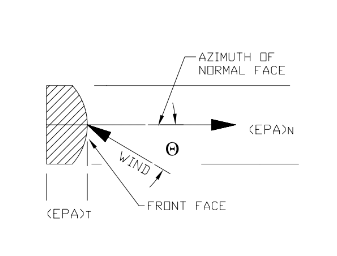
$K_a$ stands for shielding which we can apply only when the wind force considered on the back side of the antenna. We can use shielding value as recommended by TIA-222-G. In our case the permanent antenna supporting pole will shield the 3rd antenna as it is within 4D. We can use linear interpolation between 100% shielding and no shielding. We can agree that the shielding value might be around $\frac{2}{3}$, thus 66%. See on Figure 5.

$$EPA_{A3} = 2 \cdot [ 1.243 \cdot 0.623 \cdot \cos^2{(60)} + 0.543 \cdot 0.874 \cdot \sin^2{(60)} ] + \frac{2}{3} \cdot 1.243 \cdot 0.820$$
$$EPA_{A2} = 1.77 m^2$$ By having the total effective projected area of the antennas we can compute to derive the forces on the antennas multiplying with the peak velocity pressure. I show the result with the computed $c_sc_d$ factor and $c_{prob}$ factor taken into account. $$F_{w,3 antennas} = EPA_{A3} \cdot q_{p,e}$$
With $c_sc_d$ and $c_{prob}$ considered:
$$ F_{w,3 antennas} = 1.77 \cdot 1.305 \frac{kN}{m^2} = 2.310kN$$
Distribution of antenna load
The wind forces on the antennas shall be distributed to the FEA model as per Figure 6.

2-antenna configuration
The 2-antenna configuration will be similarly computed as the 3-antenna configuration. In this case I will provide also a small script to compute different scenarios for different azimuths. We assume that the 2 antennas are facing to the wind with the same azimuth, or with other words, they are mirrored to a vertical plane which goes through the supporting pole.
$$EPA_{A2} = 2 \cdot [ 1.243 \cdot 0.623 \cdot \cos^2{(\theta)} + 0.543 \cdot 0.874 \cdot \sin^2{(\theta)} ] $$
In this case the antenna back side is not computed into the projected area. However, all other accessories have to included in the FEA model (feeders, structural members, etc.) in the same way as in the 3-antenna scenario. The following code snippets shows the calculated values for the projected areas and wind forces on the antennas for several azimuth values.
Rooftop wind speed-up effect
It is not normative in Europe to consider speed-up effects on buildings. These effects need to be considered only for abrupt changes on the terrain, and define the orography factor coco for the building. However, speed-up occurs on tall buildings in real life. The speed-up effect depends on several factors, like building height and height of the parapet if any. The building needs to protrude above the immediately adjacent buildings by at least 15m. When we calculate pressure coefficients on buildings, we can see the higher pressure zones on the different segments of the building in question. These pressure coefficients in the higher zones can be in the range of 1.6 and 2.2. One can imagine if a roof-top pole is located within one of these zones then the acting wind pressure on the structure is exposed to exaggerated wind pressure. See illustration on Figure 9.
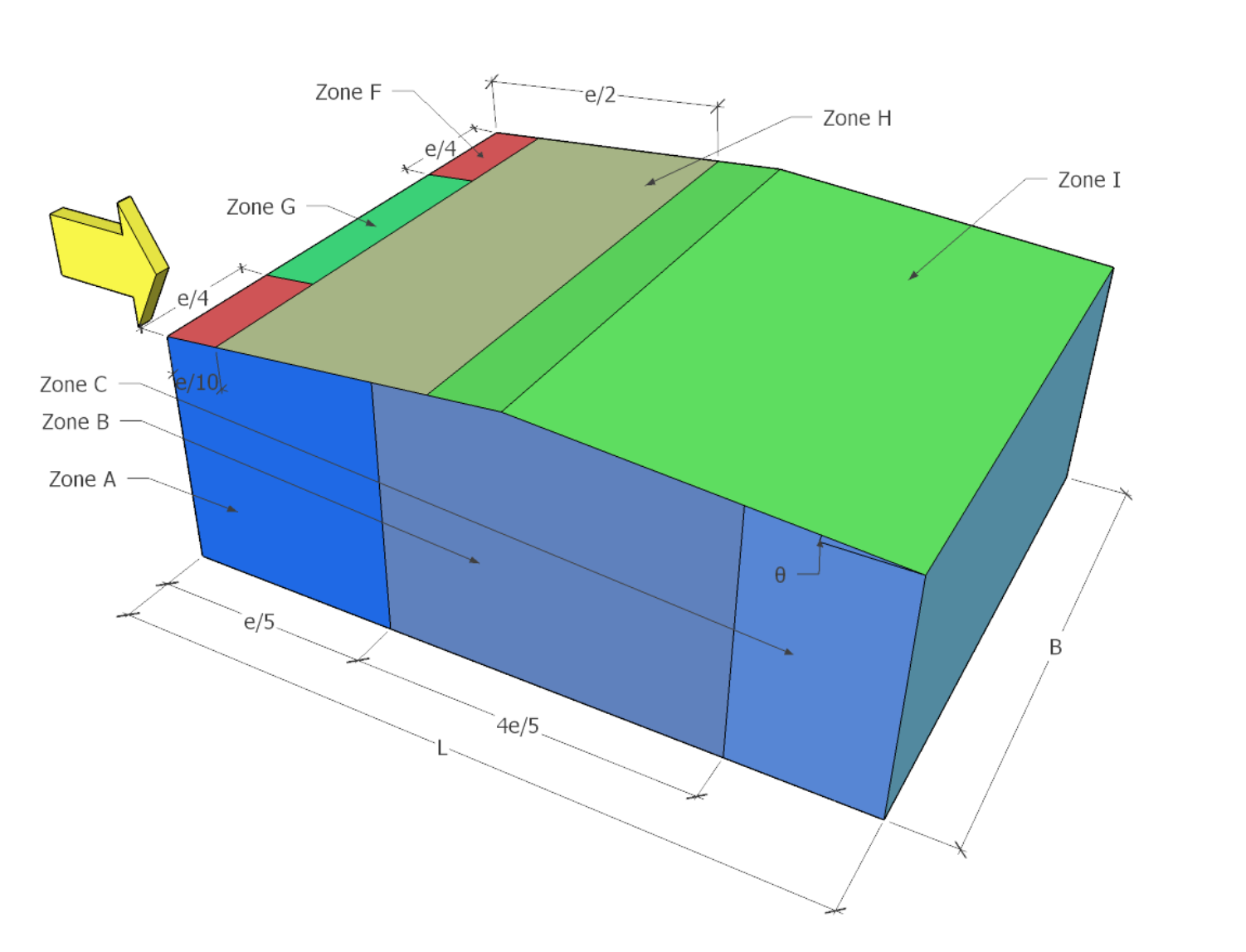
TIA-222-H as the latest version of the code demonstrate a solution for roof-top antenna supporting structures how the speed-up effect shall be defined. See more details on Figure 10. Until an implementation into the Eurocode series we suggest to take into consideration to evaluate these effects when it comes to tall buildings.

Conclusion
As you can see it is a fairly long and complicated process to establish the wind forces on antennas according to EN 1991-1-4. Certanly we can simplify some of the steps by approximating natural frequency to eliminate a dynamic analysis, or using cscdcscd factor as 1.0. But the later one doesn't really conservative for slender structures, or structures where we expect more dynamic response to the wind along-side. Like in our case, a value of 1.0 for cscdcscd would result an under designed structure.
In my opinion is that as long as Eurocode is evolving in a similar fashion in the future, we, practising engineers will need more tools to cope with these type of challenges.
Would you like to avoid making this calculation by hand? We can help you to automate these type of procedures. Get in touch with us today.